e will begin by
looking at basic biological and chemical concepts which describe biochemical
transformation of organic compounds in general and organic contaminants in
specific. These concepts include requirements for biotransformation, microbial
cell structure and function, oxidation-reduction potential, biodegradation
kinetics and bioavailability. Once these basic principals have been covered we
will concentrate on the applying them to the bioremediation of a broad range of
environmental contaminants including petroleum hydrocarbons, chlorinated
organics, nitroaromatics, heavy metals and radionuclides.
Begin by reading Chapter 4 Wiedemeyer pages 162
-169. Pay particular attention to section 4.1, Biological Fate of Contaminants.
Along with section 4.1 please refer to the rest of the text shown for session
II-1 (approximately 17 pages of text and figures) which is intended to provide
additional insight into the biotransformation/biodegradation process, the
microbial cell, enzymatics, and genetic principals. The material in session II-1
forms the basis for understanding the biotransformation process at the scale
where substrate (contaminant), electron acceptor, and other growth nutrients
interact the surface of the microbial cell.
Biotransformation/Biodegradation vs. Bioremediation.
Requirements for biodegradation.
- Microorganisms: A sufficient population and an appropriate type.
Just as a few million bacterial cells are hopelessly armed to tackle the
rigors of cleaning up a contaminated site of several hundred cubic feet in
size, so too is a single cluster of a dozen organisms on the surface of a soil
grain unsuitable for cleaning up the water flowing through nearby pore space. Thus, in order for microbial biotransformation to really
"make a difference", the microbial population must have grown
sufficiently in size to efficiently "feed" on the chemical. In
addition, if the microbes at the site are unable to transform the specific
chemicals of concern, then even in large numbers, they will be unable to exert
significant impact for site cleanup. Thus, the appropriate enzymatic machinery
targeted towards transforming the chemical of concern is required. In some
cases, where the chemicals of concern are unusual xenobiotics that are unlike
anything that exists in the natural environment, bioaugmentation is required.
- Energy & Carbon Source:
The contaminant; not usually a problem!
A source of energy (e.g., provides ATP during its biotransformation to drive the
cell's biological machinery) as well as a source of carbon (remember, the cell's
key macronutrient is carbon--see Nutrients below) are required for both
cell function (anabolism) and cell growth (catabolism).
- Contaminant Bioavailability:
Aqueous phase contaminant.
It is often assumed that a chemical (or any nutrient, for that matter) must be
present in the aqueous phase in order to be biotransformed. The reasoning behind
this assumption is simple--microbes are aquatic organisms that depend on the
diffusion of substances across their membrane from their aquatic environment.
Thus, any nutrient present in a soil, NAPL, or air phase may not be directly
accessible by microbes, and thus, is not bioavailable. Bioavailability is
governed not only by the quantity of nutrient present in the aqueous phase (low
aqueous phase concentrations may result in a phenomenon known as
"concentration-limited bioavailability"), but also by the rate of mass
transfer from nonaqueous to aqueous phases (slow rates of mass transfer from
nonaqueous to aqueous phases may result in a phenomenon known as "mass
transfer-limited bioavailability"; for example, slow rates of chemical
desorption from a soil particle may result in a condition known as "desorption-limited
bioavailability," a special case of mass transfer-limited bioavailability).
- Electron Acceptors:
e.g., O2, NO3, SO42-,
CO2
A biological transformation reaction is a special type of reaction known as an
oxidation-reduction (or "redox") reaction. Such reactions are
characterized by the transfer of electrons from more oxidized substances to more
reduced substances. In a typical reaction where a hydrocarbon such as benzene is
oxidized to catechol under aerobic conditions, benzene acts as the electron
donor and oxygen acts as the electron acceptor. In the absence of an electron
acceptor, a redox reaction will not proceed.
- Nutrients
The "chemical formula" for a microbial cell is C5H7O2NP0.083;
thus, it is clear that required nutrients for cell growth include carbon (see #2
above), hydrogen (from chemical hydrocarbons or water), and oxygen (usually from
water or the electron acceptor).
"The Primary Nutrients": N and P. In addition, the primary
nutrients of nitrogen (N) and phosphorous (P) are required. These nutrients
are often required in forms that are not part of the carbon source or electron
acceptor.
Other "Macronutrients": Ca, Mg, and Fe Calcium (Ca), magnesium
(Mg), and iron (Fe) are also required for cell metabolism. They are termed
"macronutrients" because they are often present in sufficient
quantities in soils such that Ca/Mg/Fe supplements are not needed to facilitate
biotransformation (i.e., the macronutrients usually do not limit
biotransformation rates).
Trace nutrients (i.e., trace metals). Trace metals (e.g., Cu, Zn,
Al, Vd, Mb, etc. etc.) are termed as such because (1) they are present only in
small quantities in soils, and (2) very small amounts are required for cell
function. Often, excess concentrations of trace metals can be toxic to microbes
and inhibit biotransformation (unlike excess concentrations of primary and
macronutrients, which may not necessarily be toxic to microbes). Trace metal
"pollution" is particularly troublesome where organic chemicals (which
are degraded by microbes) are co-deposited with toxic metal wastes, as might be
the case when a mine waste leachate reaches a plume of organic solvent used in
the cleaning of mining machinery. A more common circumstance occurs when organic
detergent solutions are used to clean machinery parts, floors, etc., in metal
refineries, and then discarded down a floor drain, eventually reaching the soil
environment.
- Acceptable Environmental Conditions:
Temperature, pH, and
substrate concentration.
Even if you have all of the ingredients for making a chocolate cake in a baking
pan, you won't get a perfect cake until the environmental conditions are correct
( i.e., high temperature and low ambient humidity). The same goes for
contaminant biotransformation. Nutrients, chemicals,
microorganisms, and electron acceptors provide the necessary ingredients for
redox reactions to proceed, but without the appropriate environmental conditions -- moderate temperature, moderate pH, and moderate substrate
concentrations -- biotransformation will occur very slowly or not at all.
Of particular importance is the substrate concentration. It must be sufficiently
high enough to induce enzymatic activity -- we call this concentration the threshold
substrate concentration, often denoted by Smin. Alternatively,
the chemical must not be at a concentration high enough to induce adverse
biological behavior through some type of toxicity mechanism. We call this
maximum concentration Stox.
Thus, for a substrate concentration S such that Smin < S < Stox,
and for moderate temperatures (i.e., 20-40 deg C) and pH (5-9), the
biotransformation process will proceed normally. Of course, each specific
microbial population will have specific conditions (defined by optimum values of
S, T, and pH) where biotransformation will proceed at a maximum rate for that
particular population.
The Microbial Cell: Membrane Structure and Metabolism
- Cell Structure. In general, most living cells are quite
similar. As shown in Figure II-1, they have a cell wall, which may be either a
rigid or flexible membrane. If the cell is motile, it may possess flagella
or some other hair-like appendages serving a propulsion. The interior of the
cell contains a colloidal suspension called the cytoplasm, containing
carbohydrates, proteins, and other complex organic compounds that
participate in normal cell function.
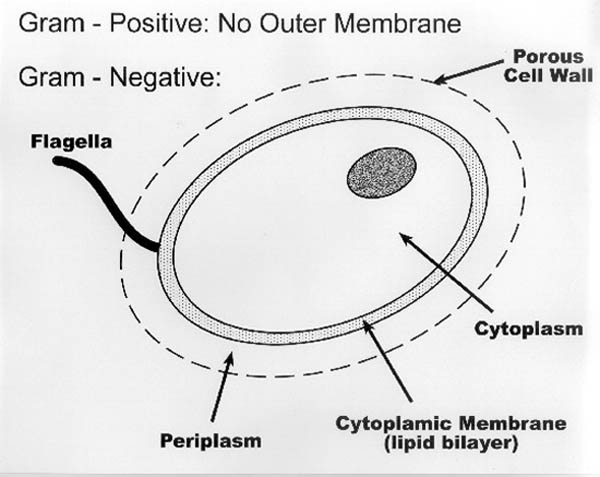
|
Figure II-1. Cellular Membrane
and Wall Structure |
|
Each cell contains nucleic acids, the hereditary material that is vital to
reproduction. The cytoplasmic area contains ribonucleic acid (RNA), whose
major role is in the synthesis of proteins. Also within the cell wall is the
area of the nucleus, which is rich in deoxyribonucleic acid (DNA). DNA
contains all the information necessary for the reproduction of all the cell
components and may be considered to be the blueprint of the cell. In some
cells, the DNA is enclosed by a membrane and the nucleus is clearly defined
(eucaryotic cells). In other cells, the nucleus is poorly defined (procaryotic
cells, which include bacteria).
- The Cell Membrane: The bacterial cell membrane (Figure II-2) is
a fluid, dynamic membrane that is composed primarily of a cytoplasmic
membrane made up of a lipid bilayer structure of phospholipids (Figure II-3).
In addition, gram-negative bacteria have an "outer" membrane that
includes lipid bilayer structure harboring a variety of functional proteins,
enzymes, lipoproteins, and polysaccharides (Figure II-2). Between the
gram-negative outer membrane and the cytoplasmic membrane, a film of fluid
called the periplasm exists. The periplasm is a common location for plasmids
that contain the genes that produce the enzymes that regulate the
transformation of a variety of environmental contaminants.
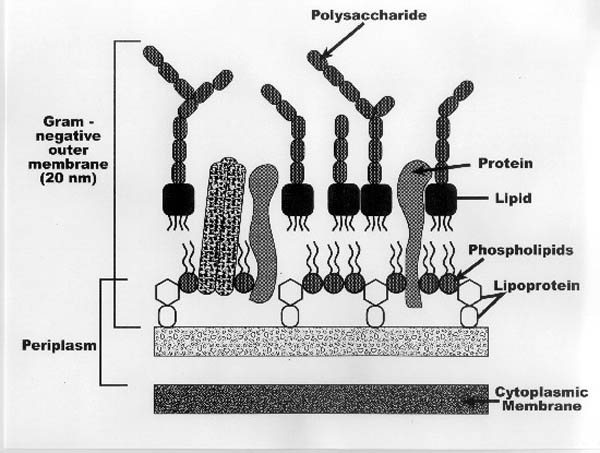
|
Figure II-2. Cross-Section of a
Gram-Negative Bacterial Cell |
|
- The Cytoplasmic membrane:
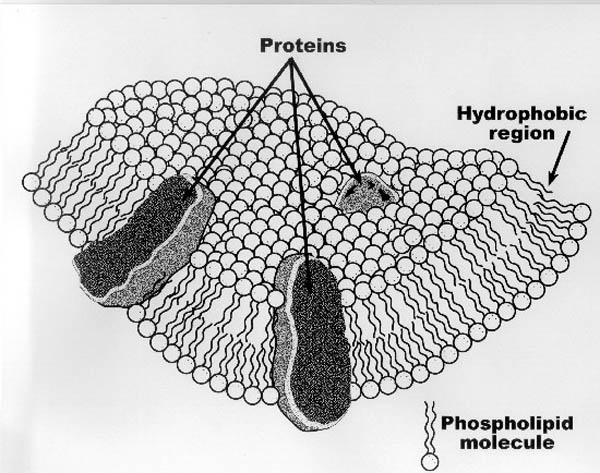
|
Figure II-3. Cross-section of the
cytoplasmic membrane showing the phospholipid bilayer
structure and membrane-bound proteins. The interior wall
(exposed to the cell's cytoplasm) and exterior wall (exposed
to either the periplasm--for gram negative cells--or the
environment--for gram positive cells) are hydrophilic
(water-loving) in nature, while the interior of the membrane
is hydrophobic. This hydrophilic-hydrophobic structure is
the root of the term "bilayer", and forms when the
hydrophobic ends of the phospholipids orient themselves
toward each other in this "lamellar" structure.
This structure is further detailed in Figure II-4. Proteins that are relatively hydrophobic will tend to orient
themselves across the membrane as shown in the figure, since
by the nature of their hydrophobicity, are attracted to the
hydrophobic region of the membrane. Hydrophilic proteins and
other charged substances, on the other hand, may be attached
the membrane's hydrophilic surfaces. |
|
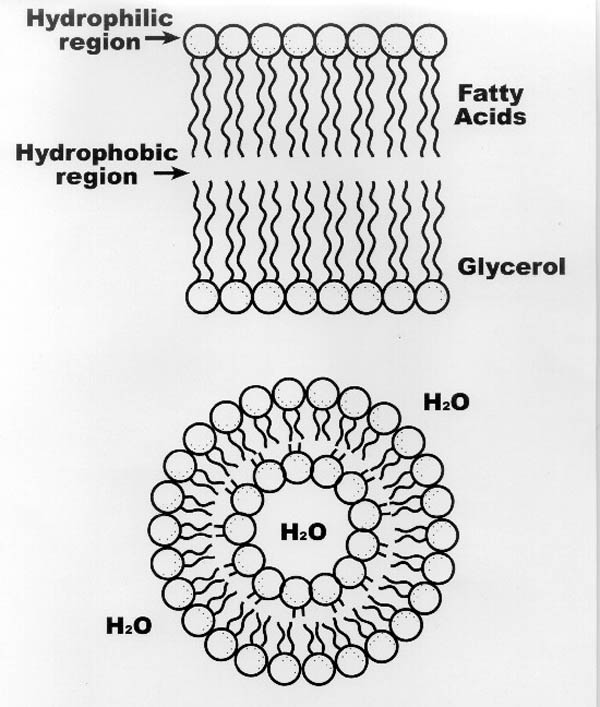
|
Figure II-4. (a) Fundamental
Structure of the phospholipid bilayer. (b) Phospholipid
membrane "vesicle"--a stable arrangement of lipids
in water. "Curly tails" on the phospholipid
molecules shown in the diagram indicate the hydrophobic
chains of the lipid (the -"lipid"), the large
circles indicate the hydrophilic glycerol groups on the
phospholipid, and the small circles indicate the hydrophilic
phosphate terminal group on the molecule (the "phospho"-). |
|
- Energy and Carbon Sources: To continue to normal function
(reproduction and other cell functions), a microbial cell must have a source
of energy and carbon for the synthesis of new cellular material. Inorganic
elements, such as nitrogen and phosphorus (the "primary
nutrients"), calcium, sulfur, potassium, magnesium, and iron (the
"macronutrients"), and other trace nutrients, are also vital to
cell synthesis. Two of the most common sources of cell carbon for
microorganisms are carbon dioxide (dissolved in water from equilibrium with
the atmospheric CO2 and/or a subsurface carbonate buffer system), and
organic matter.
- If an organism derives its cell carbon from carbon dioxide, it
is called autotrophic
- If an organism derives its cell carbon from organic carbon, it
is called heterotrophic.
The energy source (electron donor) supplies electrons to the cell in
3 stages
- Removal of electrons from the donor (oxidation)
- Transfer of electrons through one or a series of electron
carriers
- Addition of electrons to the terminal electron acceptor
(reduction)
Electron transport systems consist of a series of electron carriers
(i.e., NAD+, NADP+ ). Some electron carriers are fixed
in membrane structures (e.g., analogous to the trans-membrane proteins shown
in Figures II-2 and II-3), while others are freely diffusible, transferring
electrons to various parts of the cell. A simplified view of cell metabolism
is shown in Figure II-5.
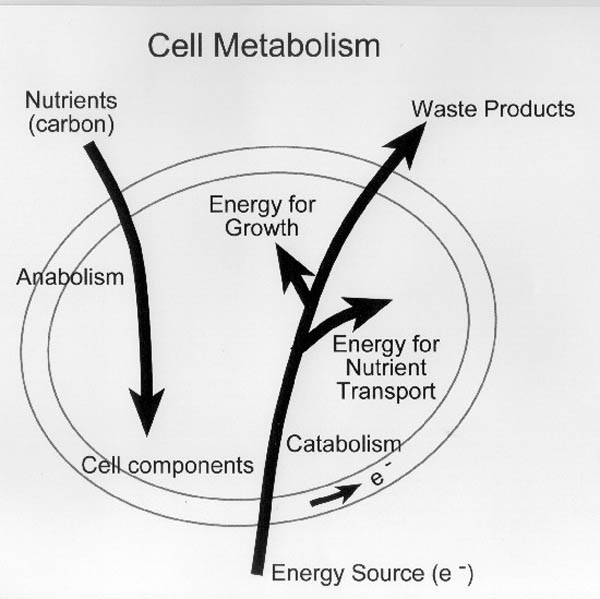
|
Figure II-5. Conceptual diagram
of cell metabolism. Cells must have a supply of carbon and
energy (electrons) to maintain their metabolism.
Carbon/energy may be supplied from a single substrate (i.e.,
glucose, benzene) or from separate sources (i.e. CO2 for
carbon and H2 as an electron donor). |
|
- Anabolism is the process by which a cell uses energy to use carbon and
nutrients for biosynthesis (the manufacture of cellular components,
including new cells).
- Catabolism is the process by which a cell transforms an energy source
(electron donor) into usable energy that can be used to facilitate
normal cell function.
Keep in mind that catabolic reactions that create energy are required to
carry out ALL cell functions, including anabolism.
A cell can catabolize without anabolism,
but a cell cannot anabolize without catabolism.
Summary of the biotransformation reaction.
- The requirements for a biotransformation reaction include a substrate (a
carbon and energy source/electron donor), and electron acceptor, cellular
nutrients, and microorganisms with the proper enzymatic machinery. Products
of the biotransformation reaction include products of cell anabolism
(biosynthesis of new cells, proteins, etc.) and byproducts of the parent
compound that was biotransformation (called reaction end points or
intermediates).
Enzymatic Catalysis of Biotransformation Reactions
- Biotransformation defined: Alteration of the chemical structure of
a compound by enzyme(s) produced by microorganism(s) such that new
compound(s) are formed. See Figure II-6.
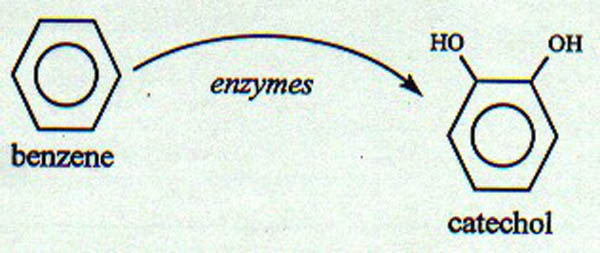
|
Figure II-6. Conceptual diagram
of the biotransformation of benzene to catechol, as mediated
by microbial enzymes. |
|
-
The activation energy must first be overcome before a
chemical reaction can proceed. A catalyst may decrease the amount of
activation energy required for the reaction to occur. Catalysts produced by
microorganisms that decrease the activation energy are called enzymes.
-
Enzymes are large protein molecules which have a high degree of
specificity for particular reactions. This specificity results from the
three-dimensional structure of the enzyme that result in its ability to act
as the "key" for a particular reaction "lock".
-
While many chemical reactions (such as the transformation of benzene to
catechol) may occur in the absence of microorganisms, some chemical reaction
rates are imperceptibly observed; enzymes typically increase reaction rates
by 108 to 1020 times the abiotic reaction rate.
Examples of Enzyme-Mediated Reactions:
- Aerobic Biotransformation of Benzene to Catechol The biotransformation of
benzene in an aerobic environment is catalyzed by an enzyme called an "oxygenase",
which put simply, is an enzyme that uses molecular oxygen to oxidize a
molecule, with the products usually being a more oxidized molecule (e.g.,
catechol) and hydrogen. Refer to Figure II-7.
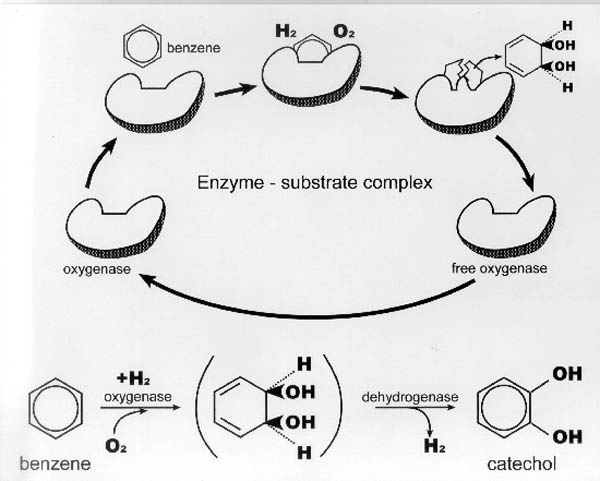
|
Figure II-7. Enzymatic catalysis of the
biotransformation of Benzene. |
|
The biotransformation of benzene to its byproducts (catechol) is mediated by
the oxygenase enzyme. Note in the figure the conceptual presentation of
the enzyme-substrate complex, with the enzyme's "shape" uniquely
suited to this particular substrate. This is a common feature of enzymatic
reactions and is a key feature of what we call "reaction
specificity".
Genetic Principles
So where do enzymes come from? Enzymes don't just magically appear.
They must be manufactured by the cell. But how does a cell know what enzymes to
make? Let's look at this process in more detail.
Let's now examine how the biotransformation process is controlled by
basic genetic principles by answering the following question: How is
biotransformation controlled by genes, DNA, RNA, and enzyme production?
The hereditary information of every cell in all organisms is carried by
molecules of deoxyribonucleic acid (DNA). These molecules are composed of two
long chains (the "double-stranded helix") containing four kinds of
building blocks, called nucleotides. The arrangement (and particularly, the
order) of these nucleotides represents a "code", which tells the cell
how to construct proteins.
Two key steps in the building of a protein are the transcription of the
genetic code from DNA to RNA (ribonucleic acid), and then its translation into a
sequence of polypeptides that make up a protein molecule.
Enzymes are a type of protein, and this is how they are made. Enzymes are
large protein molecules that act as catalysts for most of the chemical reactions
that take place in living organisms. For example, some bacteria can break down
naphthalene to salicylate through a process of six reactions, each catalyzed by
a specific enzyme. Similarly, salicylate can be broken down to pyruvate by
another seven enzymatic reactions (Figure II-8).
The sequence of enzymes involved in the degradation of naphthalene--and the
corresponding sequence of genes that control their formation--is well known. The
genes, labeled nah A, nah B, and so forth, occur in two discrete sets. The first
set is called the nah 1 operon; the second is called the nah 2 operon.
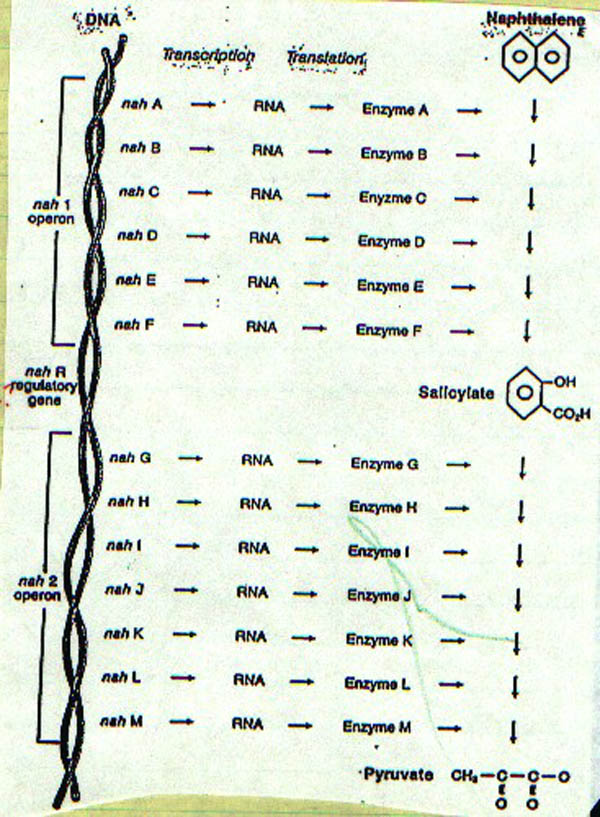
|
Figure II-8. Gene sequences for
enzymes responsible for naphthalene biotransformation. |
|
Thus, in summary:
- Gene sequences, or operons, are coded in a cell's DNA
- These codes are used to transcribe (replicate) DNA into RNA
- RNA is used to translate (build) proteins (enzymes)
- Enzymes are used to catalyze biotransformation reactions
For the breakdown of many other organic materials by bacteria, such detailed
gene sequences have not yet been identified.